Algal toxins can cause problems in the freshwater aquaculture of both vertebrates (fish) and invertebrates (shellfish). Such problems include:
- off-flavor (Tucker, 2000; SRAC Publication No. 192),
- indirect toxicity through changes in water quality (SRAC Publication No. 466), or
- direct toxicity.
Algal toxins are organic molecules produced by a variety of algae in marine, brackish and fresh waters, as well as on wet soils (Falconer, 1993). They are a problem in aquaculture when they are produced in sufficient quantities, with sufficient potency, to kill cultured organisms, decrease feeding and growth rates, cause food safety issues, or adversely affect the quality of the product (Shumway, 1990).
Algal Blooms
The production of algal toxins is normally associated with algal blooms, or the rapid growth and exceptionally dense accumulation of algae. The term Harmful Algal Bloom (HAB) is used to describe a proliferation of algae, or phytoplankton. Severe blooms of even non-toxic algae can spell disaster for cultured animals, because blooms deplete the oxygen in the shallow waters of many aquaculture systems. The number of HABs around the world is increasing (Shumway, 1990; Sunda et al., 2006), especially in the U.S. where almost every coastal state is now threatened, in some cases by more than one species of harmful algae. Scientists are unsure why this trend is occurring. The causes may be natural (species dispersal) or human-related (nutrient enrichment, climate change, and/or transport of algae in ship ballast water) (Johnk, et al. 2008; Sunda et al., 2006).
The effects of algal blooms vary widely. Some algae are toxic only at very high densities, while others can be toxic at very low densities (a few cells per liter). Some blooms discolor the water (thus the terms “red tide” and “brown tide”), while others are almost undetectable with casual observation (Shumway, 1990).
HABs can affect public health and ecosystems when:
- filter-feeding shellfish (clams, mussels, oysters, scallops) feed on toxic phytoplankton and accumulate harmful toxins that are passed up the food chain;
- fish, shellfish, birds and even mammals are killed by eating organisms that have consumed algal toxins;
- light cannot penetrate the water, thus changing the function and structure of the aquatic ecosystem;
- discoloration makes water aesthetically unpleasant;
- the decaying biomass of a bloom depletes dissolved oxygen (especially critical in aquaculture); or
- blooms kill other algae important in the food web (Codd et al., 2005b; Landsburg, 2002).
HABs can cause serious economic losses in aquaculture if they kill cultured organisms or cause consumers concern about food safety. Preliminary estimates show that the effect of HAB outbreaks on the U.S. economy is more than $40 million per year, or $1 billion per decade (Landsburg, 2002; Hudnell, 2008).
Toxin-producing algae may become more prevalent in the future (Sunda et al., 2006; Johnk et al., 2008), especially in eutrophic freshwater systems. This publication focuses on algal toxins in freshwater pond aquaculture in the South and southeastern United States. The most common toxin-producing algae in this region are cyanobacteria, golden algae (Prymnesium parvum) and euglenoids.
Cyanobacteria: The Blue-Green Algae
Cyanobacteria (blue-green algae) inhabit fresh, brackish, marine and hypersaline waters, as well as terrestrial environments. Cyanobacteria grow in many habitats from thermal springs to the arctic. They play important roles in the biogeochemical cycling of elements and in the structure, function and biodiversity of aquatic communities (from microbes through vertebrates). Some cyanobacteria can reduce both N2 and CO2. Some can convert N2 into NH3 and, ultimately, into amino acids and proteins.
Cyanobacteria have a relatively simple prokaryotic structure and lack membrane-bound organelles (nucleus, mitochondria and chloroplasts). With murien in the cell wall and reproduction by binary fission, cyanobacteria are structurally and physiologically like other gram-negative bacteria, but they conduct photosynthesis like plants in aquatic systems. Cyanobacteria are much larger than other bacteria and make a major contribution to world photosynthesis and nitrogen fixation (Codd et al., 2005a; Huisman et al., 2005; Hudnell, 2008).
Cyanobacteria occur in unicellular, colonial and filamentous forms and most are enclosed in a mucilagenous sheath, either individually or in colonies. As single cells, large colonies and filaments (trichomes), blue-green algae can become the dominant algae in nutrient-rich waters. They can form blooms so thick it appears that blue-green paint covers the surface of the water.
Several species found in the South and Southeast produce substances that cause taste and odor problems in water supplies and aquacultural products (Tucker, 2000). Some blue-green algae, particularly Anabaena and Microcystis, produce toxins poisonous to fish and to wildlife and livestock that drink contaminated water. There are also documented cases of blue-green algal toxins harming people in other parts of the world who drank poorly treated water.
Cyanobacterial Ecology in Aquaculture Ponds
Cyanobacteria can colonize and rapidly grow to great masses in aquaculture ponds. Factors that affect their growth are nutrient status, salinity or ionic strength, light conditions, turbulence and mixing, temperature and herbivory (Sunda et al., 2006). In aquaculture situations, eukaryotic algae (green, diatoms, etc.) can often grow faster than cyanobacteria. However, cyanobacteria can out-compete algae for nutrients, thrive with low dissolved oxygen, and photosynthesize more efficiently at low light levels. Cyanobacteria are less affected by turbidity, high concentrations of ammonia and warm temperatures. They can seize the advantage in eutrophic aquaculture situations. Cyanobacteria can affect the production of zooplankton and consequently the production of fish. They also produce allelochemicals that can inhibit competing algae and invertebrate grazers (Gross, 2003; Berry et al., 2008).
There is compelling evidence that cyanobacteria and their toxins (both neurotoxins and hepatotoxins) affect zooplankton (cladocerans and rotifers) population structure, and that this may influence the ecological processes responsible for cyanobacterial success (Berry et al., 2008). Zooplankton generally avoid cyanobacteria as a food source (Gross, 2003), which means that zooplankton feed on algae that compete with cyanobacteria. In the process, they release essential nutrients, further fertilizing cyanobacterial growth. During cyanobacterial blooms, when alternative food sources for zooplankton have been exhausted, Daphnia populations may decline. Some zooplankton (Daphnia pulicaria, Daphnia pulex) species have adapted to survive in the presence of certain toxic cells (Sunda et al., 2006; Gross, 2003). This changes the zooplankton population dynamics. Feeding pressure by adapted zooplankton on cyanobacteria is reduced by fish predation, which again releases nutrients that fuel cyanobacterial growth. It may be premature to propose that cyanobacterial dominance is guided by cyanotoxin production. However, feeding deterrence is one of the roles suggested for these metabolites (Berry et al., 2008). Whether the compounds causing toxicity and deterrence are one and the same has recently been questioned (Berry et al., 2008). While daphnids are killed when feeding on toxic Microcystis cells, they show no selection between ingesting toxic or nontoxic cells, which indicates that microcystins are not responsible for feeding inhibition (Berry et al., 2008).
Problems with Cyanobacteria in Aquaculture Ponds
Cyanobacteria can rapidly overtake an aquaculture pond and contribute to unstable conditions. Cyanobacteria blooms can decrease fish production and kill fish because of oxygen depletion. Cyanobacteria can also cause off-flavor and objectionable odor in fish.
However, the role of cyanobacteria and cyanotoxins in fish kills and other problems is not clear at this time. There are more than 1 million fish ponds in the Southeast and many of them have relatively frequent blooms of cyanobacteria that may produce toxins (e.g., Microcystis, Anabaena, etc.). Yet there are only a few reports of fish kills that are directly related to algal toxin production (Zimba et al., 2001). So the mere presence of toxin-producing algae does not necessarily mean that enough toxin will be produced to harm fish in culture.
Cyanobacterial Toxins
Cyanobacterial toxins can be classified several ways. They may be classified according to their chemical structures as cyclic peptides (microcystin and nodularin), alkaloids (anatoxin-a, anatoxin-a(s), saxitoxin, cylindrospermopsin, aplysiatoxins, lyngbyatoxin-a) and lipopolysaccharides. However, cyanotoxins are more commonly discussed in terms of their toxicity to animals. While there are several dermatotoxins (e.g., lyngbyatoxin and aplysiatoxins), which are produced primarily by benthic cyanobacteria, most cyanotoxins are either neurotoxins or hepatotoxins (Codd et al., 2005a).
Neurotoxins. Neurotoxins are organic molecules that can attack the nervous systems of vertebrates and invertebrates. Three primary types of neurotoxins have been identified: 1) anatoxin-a, an alkaloid, inhibits transmissions at the neuromuscular junction by molecular mimicry of the neurotransmitter acetylcholine (blocks post-synaptic depolarization); 2) anatoxin-a(s) blocks acetylcholinesterase (similar to organophosphate pesticides); 3) saxitoxins are carbamate alkaloids that act like carbamate pesticides by blocking sodium channels.
Neurotoxins are produced by several genera of cyanobacteria including Anabaena, Aphanizomenon, Microcystis, Planktothrix, Raphidiopsis, Arthrospira, Cylindrospermum, Phormidium and Oscillatoria. Neurotoxins produced by Anabaena spp., Oscillatoria spp. and Aphanizomenon flos-aquae blooms have been responsible for animal poisonings around the world (Carmichael, 1997; Briand et al., 2003).
Neurotoxins usually have acute effects in vertebrates, with rapid paralysis of the peripheral skeletal and respiratory muscles. Other symptoms include loss of coordination, twitching, irregular gill movement, tremors, altered swimming, and convulsions before death by respiratory arrest.
Hepatotoxins. Hepatotoxins are produced by many genera of cyanobacteria and have been implicated in the deaths of fish, birds, wild animals, livestock and humans around the world (Briand et al., 2003; Carmichael, 1997). The cyclic heptapeptides, or microcystins, inhibit eukaryotic protein phosphatases type 1 and type 2A, resulting in excessive phosphorylation of cytoskeletal elements and ultimately leading to liver failure (Codd, 2005b). These toxins target the liver by binding the organic anion transport system in hepatocyte cell membranes. Microcystins are the largest group of cyanotoxins, with more than 70 structural variants (Malbrouk and Kestemont, 2006). Microcystin is the only cyanotoxin for which the biosynthetic pathway and gene cluster have been identified (Huisman et al., 2005). Microcystins are produced in fresh waters by species of Microcystis, Anabaena and Planktothrix. Symptoms of poisoning in fish include flared gills because of difficulty breathing and weakness or inability to swim. Channel catfish, Ictalurus punctatus, can become intoxicated at ~50 to 75 ?g microcystin/L (Zimba et al., 2001). All fish may be killed within 24 hours of exposure. At necropsy, severe lesions may be observed in liver tissues.
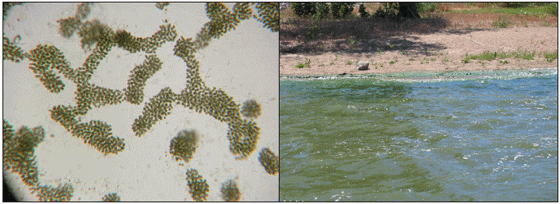
One potent hepatotoxin, cylindrospermopsin, is produced by Cylindrospermopsis raciborskii, a relatively small cyanobacterium. Cylindrospermopsin is an alkaloid that suppresses glutathione and protein synthesis. C. raciborskii has been in the South and Southeast for decades and is becoming more widespread. Mammals (such as humans) are relatively sensitive to cylindrospermopsin and may be affected when they eat fish that have been exposed to the toxin. A study reporting the bioaccumulation of cylindrospermopsin in muscle tissue of the redclaw crayfish (Cherax quadricarinatus) and visceral tissues of rainbow fish (Oncorhynchus mykiss) shows that exposure could occur from farm-raised freshwater aquatic foods. Fish are generally more tolerant of algal toxins than mammals and tend to accumulate them over time (Carson, 2000). Although C. raciborskii has not yet been a problem in aquaculture, it could become a problem in the future.
Environmental Effects on Toxin Production
The effects of environmental factors on toxin production are much studied and widely disputed (Codd, 2000; Codd et al., 2005a). Blooms in the same body of water can be toxic or non-toxic from one year to the next. A different strain composition (i.e., toxic versus non-toxic), which cannot be distinguished microscopically if belonging to the same species, is a common explanation for this occurrence. However, some species are known to produce high or low levels of toxicity under different laboratory conditions. The stimulus for toxin production in such species is not known. Environmental parameters such as light intensity, temperature, nutrients and trace metals have been mimicked under laboratory conditions and their effect on cyanotoxin production investigated. Studies on light intensity are not definitive, but it is known that intense light increases the cellular uptake of iron, which may be responsible for more toxin production. However, low concentrations of iron lead to higher microcystin concentrations (Huisman et al., 2005). Nutrients such as nitrogen and phosphorus are essential for cyanobacterial growth. Phosphorus is usually the limiting factor in ponds, so small increases in this nutrient may influence toxin production simply as a result of increasing algal growth. Generally, decreased amounts of microcystin (produced by Anabaena, Microcystis and Oscillatoria) and anatoxin-a (produced by Aphanizomenon) have been reported under the lowest phosphorus concentrations tested (Watanabe et al., 1995).
Managing Toxic Cyanobacteria
Monitoring and diagnosing the problem. While not all blooms of toxin-producing cyanobacteria result in toxin production, most do. Once a bloom is observed, the onset of toxicity will be rapid (hours to a day or two). To confirm the problem, a diagnostician will need fresh samples (unpreserved) of the water containing the suspected cyanobacteria (Rottmann et al., 1992). A sample of both sick and dead fish will also be needed, along with information on fish behavior and any other symptoms observed. Young fish are generally more sensitive than older fish. The diagnostician may look for lesions on fish livers, although this is inconclusive as the sole method of diagnosis (Zimba et al., 2001).
Treatment. Most of the time, managing a pond specifically to prevent toxic blue-green algae blooms is not justified, and the treatments themselves are risky. An algicide should not be applied without considering the size of the affected pond, the number and type of fish at risk, the age and condition of the fish, the sensitivity of the cyanobacterium to treatment, and the cost of the treatment. Non-chemical treatments include 1) physical mixing and aeration, 2) increasing flow rate or flushing to decrease hydraulic retention time, and 3) decreasing or altering nutrient content and composition. Some of these options may not be viable at all sites and in all situations.
Prymnesiophytes: The Golden-Brown Algae
The haptophyte genus Prymnesium is comprised mainly of toxin-producing species that form harmful blooms usually in brackish water (West et al., 2006). Blooms of P. parvum have been responsible for fish kills and significant economic losses in Europe, North America and other continents. Texas has been hit with recurrent blooms in several reservoirs and rivers and Texas Parks and Wildlife has offered some detailed advice regarding management options (Sager et al., 2007).
Prymnesiophyte Ecology
Prymnesium parvum is commonly called the “golden” alga. It is considered to be a haptophyte protist (Green and Leadbetter, 1994). It is a relative small (~10 ?m), generally halophilic organism that intermittently produces an ichthyotoxin. This organism has been implicated in numerous extensive fish kills in brackish waters and inland waters with relatively high mineral content on five continents (Otterstrom and Steemann-Nielsen, 1940; Holdway et al., 1978; James and de la Cruz, 1989; Kaartvedt et al., 1991; Guo et al., 1996; Lindholm et al., 1999).
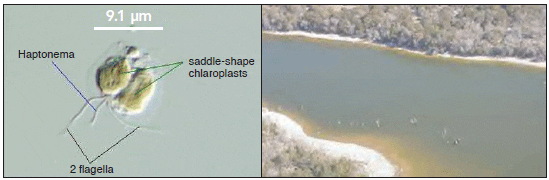
P. parvum cells contain chlorophylls a and c as well as yellow-brown accessory pigments and are capable of photosynthesis. However, the organism is thought to be a mixotroph that can feed on bacteria and protists (Skovgaard et al., 2003) and phagotrophy has been observed in cultures. P. parvum has a vitamin requirement in laboratory culture (Droop, 1954).
The Prymnesium Toxins
Prymnesium parvum produces at least three toxins—an ichthyotoxin (Ulitzer and Shilo, 1966), a cytotoxin (Ulitzer and Shilo, 1970) and a hemolysin (a protein that lyses red blood cells) (Ulitzer, 1973). These toxins are collectively known as prymnesins and all can alter cell membrane permeability (Shilo, 1971). Bloom density does not correlate strongly with toxicity (Shilo, 1981), probably because toxicity may be enhanced by temperature lower than 30 ºC (Shilo and Aschner, 1953), pH greater than 7.0 and phosphate concentration (Shilo, 1971).
The P. parvum ichthyotoxin affects gill-breathing aquatic animals such as fish, brachiated tadpoles and mollusks (Shilo, 1967). It causes gill cells to lose their selective permeability and, thus, makes them vulnerable to any toxin in the water (Ulitzer and Shilo, 1966; Shilo, 1967).
Signs of Intoxication
Dense growths of Prymnesium parvum may color the water yellow to copper-brown or rust. The water may foam if aerated or agitated. Affected fish behave erratically. They may accumulate in the shallows and be observed trying to leap from the water to escape the toxins (Sarig, 1971). Affected fish may have evident blood in gills, fins and scales and may be covered with mucus. Young fish are often most sensitive to the toxin. If fish are removed to uncontaminated water in the early stages of intoxication, their gills can recover within hours (Shilo, 1967). However, feeding and growth will usually be reduced if fish survive (Barkoh and Fries, 2005).
Aquatic insects, birds and mammals are not affected by P. parvum toxins. The golden alga is not known to harm humans either, although dead or dying fish should not be eaten.
Managing Prymnesiophytes
Monitoring and diagnosing the problem. Identifying Prymnesium parvum requires examining unpreserved, discrete water samples. P. parvum can pass through many plankton nets and their structure can be altered and distorted by preservatives or fixatives. P. parvum cells can be detected at low concentrations (~102 cells /mL) using fluorescent microscopy on live or reasonably fresh samples. P. parvum cell densities can be determined using a hemacytometer (Barkoh and Fries, 2005).
Prymnesium parvum N. Carter are small, subspherical, swimming cells about 8 to 15 ?m long with two equal or subequal heterodynamic flagella 12 to 20 ?m long and a short (3 to 5 ?m), flexible, non-coiling haptonema (Green et al., 1982). Cells have two chloroplasts that may be “c-shaped” and olive green in color. They swim with a smooth forward movement while the cell spins on the longitudinal axis and flagellar pole. P. parvum cells have diagnostic calcareous scales that can be observed with electron microscopy (Green et al., 1982). Experience is required to identify this alga.West et al. (2006) have developed a specific monoclonal antibody used in conjunction with solid-phase cytometry to rapidly quantify P. parvum.
A bioassay can be used to estimate ichthyotoxin production by Prymnesium parvum (Sager et al., 2007). This assay is useful in deciding whether to apply an algicide. The assay involves exposing fish such as larval Pimephales promelas to the pond water in question, and to dilutions of the suspect water amended with a cofactor or promoter of P. parvum toxin. Ulitzer and Shilo (1966) discovered that the potency of the P. parvum ichthyotoxin was augmented by the cation DADPA (3,3-diaminodipropylamine) in laboratory tests because it increased the sensitivity of Gambusia to the toxicant. This bioassay can identify waters that have sufficient toxin (or are developing sufficient toxin concentrations) to pose a risk to fish in culture.
For diagnostic analysis, 10-liter water samples should be collected at least 6 inches below the surface because P. parvum is sensitive to UV radiation. Both cell counts (microscopy) and the toxin bioassay are needed to confirm P. parvum, so unpreserved water samples must be shipped expeditiously to the lab.
Treatment. Texas Parks and Wildlife has detailed advice for managing Prymnesium parvum (Sager et al., 2007). One method used in isolated pond culture is applying ammonium sulfate and copper sulfate (Barkoh et al., 2003). The ammonium sulfate concentrations required to control P. parvum (~0.17 mg/L of un-ionized ammonia) may produce un-ionized ammonia concentrations that adversely affect some fish (Barkoh et al., 2004). If not carefully used, the copper sulfate may kill desirable algae along with the P. parvum and decrease food resources for the zooplankton, thereby disrupting fish feeding. Neither barley straw nor a commercial bacterial bioaugmentation product were effective for controlling P. parvum in Texas ponds (Barkoh et al., 2008). Barley straw extract was also ineffective in laboratory tests (Grover et al., 2007). Treatments with high concentrations of ammonium (0.72 mg NH4-N/L) were successful, though they pose a risk to non-target species. Repeated treatments of ammonium chloride and phosphoric acid were somewhat successful for controlling P. parvum growth in limnocorrals in aquaculture ponds (Kurten et al., 2007), but they are also hazardous to non-target species.
In the Chinese aquaculture of carp species, suspended solids (mud), organic fertilizer (manure) and decreased salinity have been used to control P. parvum (Guo et al., 1996), with the best results from decreased salinity and ammonium sulfate.
When using an algicide, obtain all regulatory approvals and permits and follow label instructions and restrictions to comply with federal law.
Euglenoids
Since 1991, several outbreaks of toxic Euglena (Fig. 3) have occurred in North Carolina hybrid striped bass (Morone saxatilis x M. chrysops) production ponds, causing the loss of more than 20,000 pounds of fish.
Relatively recent research has confirmed that Euglena species produce an ichthyotoxin in freshwater aquaculture (Zimba et al., 2004). The hybrid striped bass mortalities in North Carolina were caused by E. sanguinea, a widely distributed species in many shallow, relatively calm, eutrophic freshwater systems. This species also killed laboratory-reared channel catfish, tilapia (Oreochromis niloticus) and striped bass. In confirmatory studies, Zimba et al. (2004) noted that cultures of Euglena granulata (UTEX LB2345) caused similar mortalities and symptoms in channel catfish and sheepshead minnows (Cyprinodon variegatus).
Diagnosis
Microscopic examination of a water sample can confirm the presence of Euglena since E. sanguinea is morphologically distinct. The typical progression of symptoms from exposure to Euglena toxin begins with the fish going off feed for no apparent reason. Within 24 hours of cessation of feeding, the fish swim at or near the surface in an agitated or disorientated state, often with the dorsal fin extending out of the water or swimming on their sides and even upside down. If steps are not taken immediately after observing this state, within 24 hours the fish will be dead.
Field observation of dead fish indicated rapid onset of mortality with reddening of gill tissue. Mortality of laboratory-reared channel catfish occurred within 6.5 minutes to 2 hours after exposure. No water quality characteristics (ammonia, nitrate, pH, temperature or dissolved oxygen) were abnormal or exceptional during the fish mortalities. Fish exposed to E. sanguinea in culture or to filtrate from cultures were disoriented rapidly. Their respiration was accelerated and they lost the ability to maintain equilibrium. Although no distinct hemorrhaging was observed, gill tissue was reddened (Zimba et al., 2004).
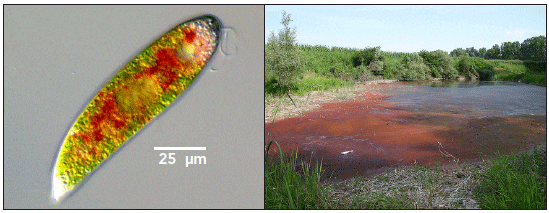
Euglena Toxin
Based on behavioral changes in exposed fish, Zimba et al. (2004) suggested that the euglenoid toxin functions as a neurotoxin. The toxin is water soluble, non-protein, heat stable (30 °C for 10 minutes), freezing stable (-80 °C for 60 days), and labile to oxidation. Euglenoids should be sensitive to several of the algicides available. If a toxic Euglena bloom occurs, try to minimize mixing of the water because mixing will disburse the bloom throughout the pond. Aerate only if needed to save the fish from acute oxygen depletion. Although Euglena sp. are normally very mobile, as a bloom progresses to the point that fish are disorientated, the algae seem to become concentrated in the downwind side of the pond.
In 2002, work by Rowan and others on a Euglena bloom at the Tidewater Research Station in North Carolina showed that potassium permanganate applied at a rate of 2.5 times the permanganate demand of the pond apparently detoxified the toxin and eliminated the source. Lower rates detoxified the toxin but did not eliminate the source, and the symptoms of agitation and disorientation recurred quickly in exposed fish. Researchers at UNC-Wilmington identified the alga as Euglena sanguinea and are attempting to isolate the toxin and study it.
Mention of a control tactic for toxin-producing algae does not constitute endorsement of an algicide or any other tactic for your specific situation. Check with your local Extension agent regarding site specific permit requirements and restrictions.